A Natural Incident in the Orbit
A mission to bring internet for all, with almost 42,000 satellites in the Earth’s orbit, was scheduled to receive 49 more satellites on 3rd February 2022 during its 36th Launch. This launch would have brought the total satellite number to around 2,091. The mission was expected to be seamless, as it had been before, until now.
Just a day after the launch, when the satellites were gradually propelling towards the 500 km orbit, they were hit by an unexpected hindrance that resulted in losing 38 satellites, leaving just two to be active. This issue was not space debris, as one would expect, but the Sun’s coronal mass ejection, which led to a geomagnetic storm, caused an estimated loss of $50 million.
Satellites falling out of orbit due to solar storms is not an isolated phenomenon; in another instance, a SAR EO company, Capella Space, have faced a similar impact, as their satellites – Capella 7 and 8, experienced premature deorbiting due to higher solar activity throughout their operation phase, as compared to Starlink’s incident that occurred even before the satellites could station themselves in the desired orbits.
This atmospheric condition based on the space environment would have been calculated per the NOAA predicted values for the mission. However, the observed solar weather was more intense than predicted. This, coupled with a supposed underperformance of the propulsion system, would have led to rapid de-orbiting.
Where Starlink was affected by a rare solar storm or a flare event, Capella experienced consistent higher-than-anticipated solar activity during the solar cycle. But the impact travels much more profound and beyond the orbit. Intense geomagnetic storms also have the potential to induce electric currents in power lines, potentially damaging transformers and other components of a power grid. This underscores the importance of studying and monitoring solar weather to mitigate potential disruptions and damages to satellite systems and terrestrial infrastructure.
Analysing the Impact – The Intensity of Solar Weather
Series of events: On 29 January 2022, Coronal Mass Ejections (CMEs) erupted from the Sun towards Earth, observed by the Solar and Heliospheric Observatory (SOHO) at the L1 point and captured by the STEREO-A spacecraft. Based on the Space Weather Forecast Office’s predictions, the solar wind plasma, travelling at approximately 500 km/second, was expected to reach Earth in 3-4 days.
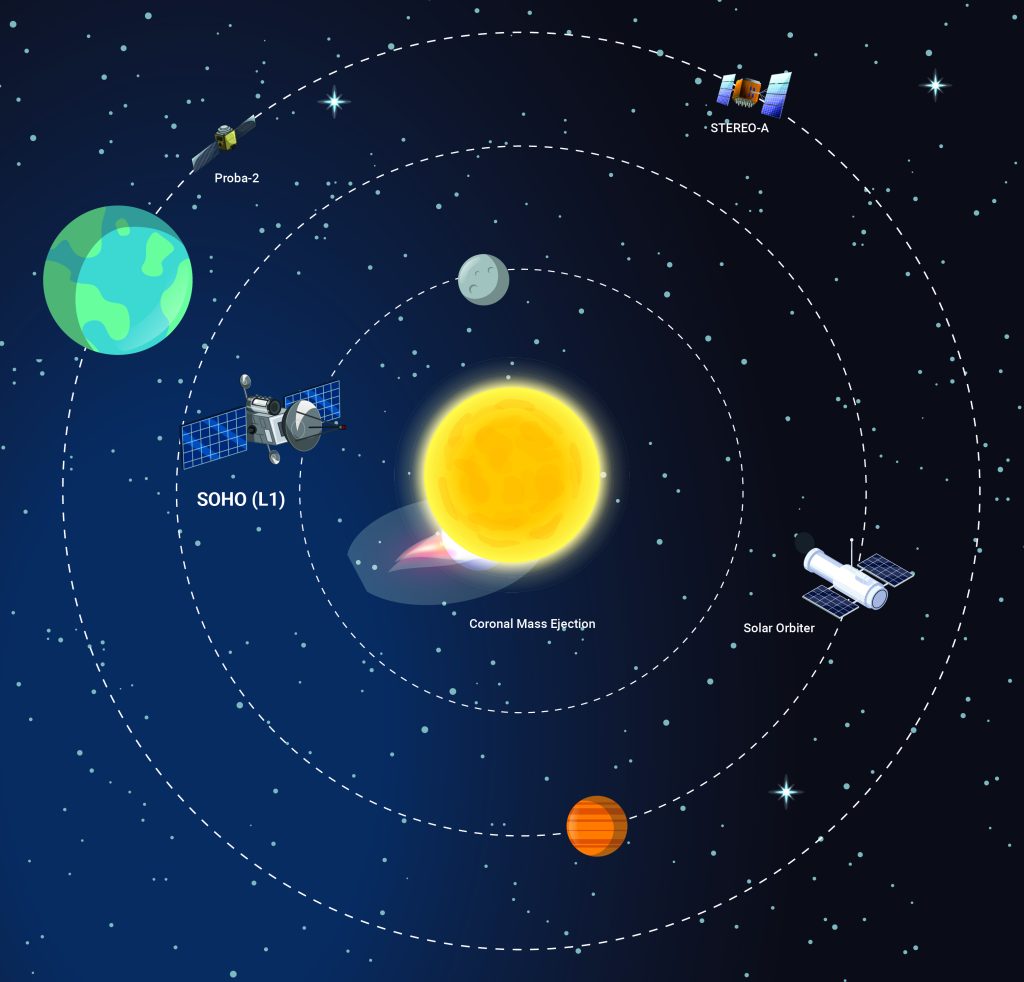
Arriving on 3rd February, the CMEs created a geomagnetic storm impacting the thermosphere and ionosphere. Observations revealed that the CME event elevated the global geomagnetic activity Kp index to 5 for 6 hours on the 3rd and 4th of February. This minor storm, as classified by SWPC’s Space Weather Scales, caused significant thermospheric and ionospheric disruptions, consistent with prior studies, which resulted in the rapid deorbiting and the loss of Starlink satellites.
In the Starlink Group 4-7 mission launched on February 3, 2022, out of the 38 lost satellites, only two had good enough tracking points to get a decaying trend, shown in Figure 1.
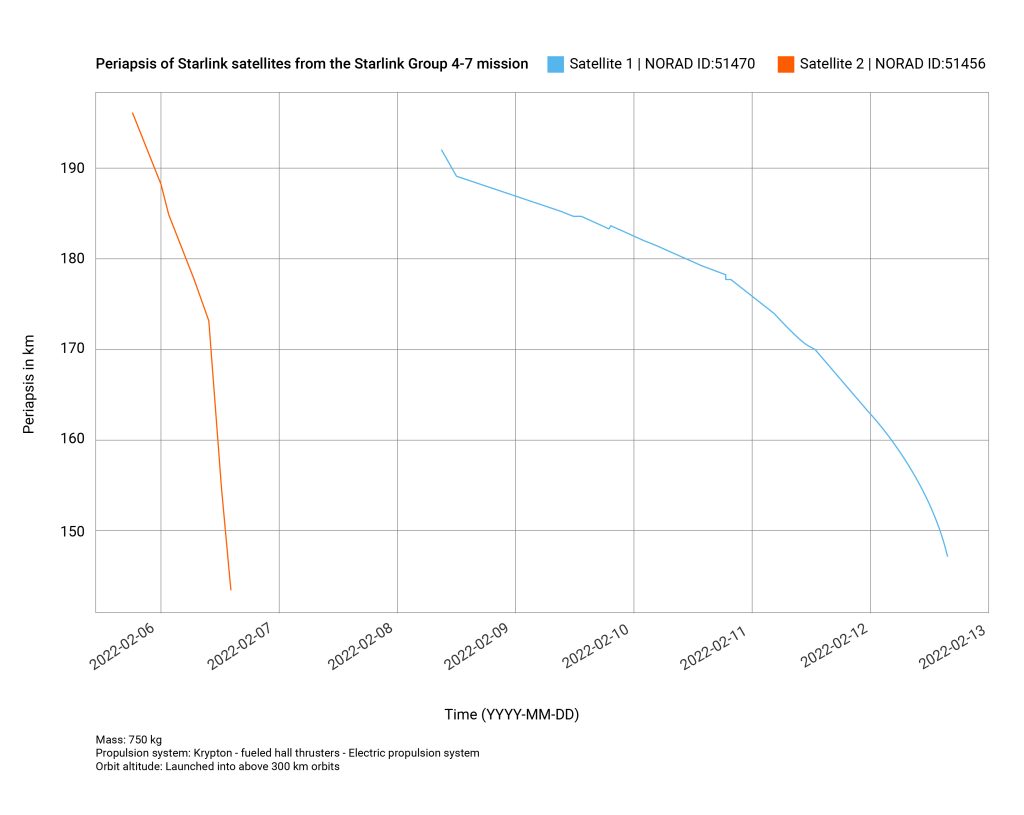
This tracking data is sourced from the openly available Space-Track website. The initial tracing data after the launch date of Feb 3 2022, is unavailable, but the launch altitude of these satellites is reported to be around 280 km by the Jonathan Space Report.
Capella constellation:
Capella constellation consists of 10 SAR EO satellites to date. Out of the ten satellites, only five are currently in orbit, of which two are already decaying more rapidly than expected. The periapsis decay trends are shown in Figure 2.
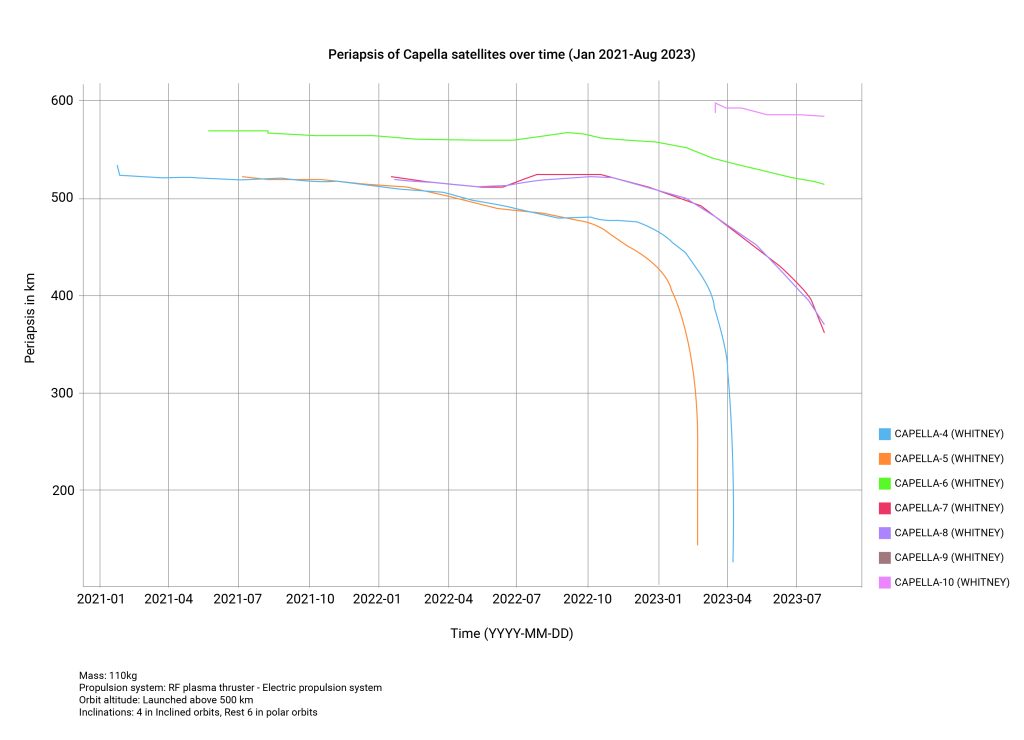
One can observe a correlation between the premature deorbiting of the Capella 7 and Capella 8 satellites and the increased solar activity during their lifetime. The intensity of solar weather is quite inversely proportional to the satellite’s orbital progress.
For instance, the increased solar activity around the same time can be observed in Figure 3, which shows the gradual deviation of the observed solar flux values from the nominal predicted values beyond the maximum bound starting from early 2021.
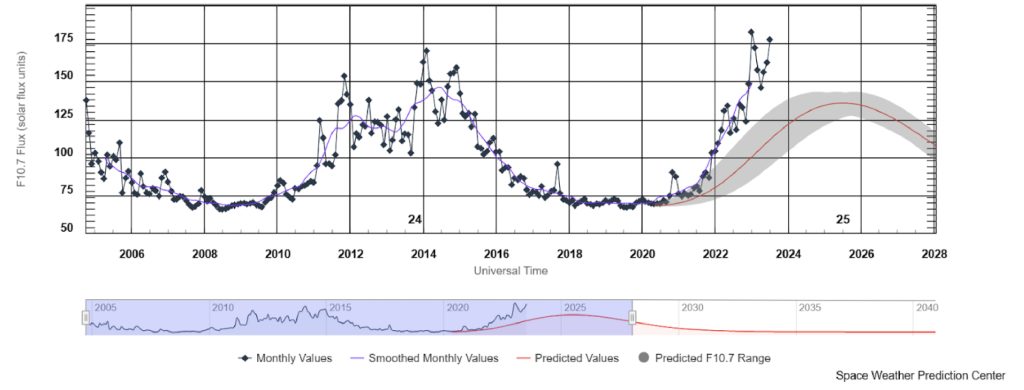
In both cases, the satellites had electric propulsion systems, and it was seen that they could not counter the increased atmospheric drag effects. These incidents highlight that the impact of atmospheric effects due to intensified solar weather is one of the most important factors to consider in designing, building and operating a satellite mission in a low earth orbit (LEO) and very low earth orbit (VLEO).
So, What is Solar Weather?
As said above, the CME impacts the Earth’s atmosphere by impacting the ionosphere and thermosphere. This leads to the expansion of the Earth’s atmosphere, impacting the satellites and resulting in higher drag. This is considered third-body perturbation forces, solar radiation pressure, and atmospheric drag.
Solar weather, also known as space weather, refers to the conditions and disturbances in the space environment surrounding the Earth caused by the Sun’s activity. Solar activities can be broadly divided into four main components:
- Solar flares
- Coronal Mass Ejections (CMEs)
- Solar wind
- Solar energetic particles.
Solar flares are intense and sudden bursts of energy and radiation from the Sun’s surface. These are caused by the rapid release of magnetic energy stored in the Sun’s atmosphere. Solar flares can be observed in a wide range of electromagnetic radiation, from radio waves to gamma rays, and can significantly impact space weather. Solar flares are often (but not necessarily) followed by the eruptions of plasma and magnetic fields from the Sun’s corona in the form of Coronal Mass Ejections or CMEs.
These eruptions expel billions of high energy charged particles (called solar energy particles) into space at extremely high speeds, ranging from tens of kilometres per second to a few thousand (near the corona in the field of coronagraphs).
The CMEs can travel over a long distance into the solar system, and in the process, they accelerate the background solar wind and can create severe solar storms. It is to be understood that solar winds are a continuous stream of charged particles originating from the solar corona that travels the solar system and, hence, becomes a primary source of solar weather. Though the CMEs have a similar origin and constitution, they are much more powerful but rare, which occurred in the case of the Starlink launch.
To effectively address such formidable solar events and ensure preparedness in satellite design and mission operations, stakeholders must adopt a proactive approach. This involves monitoring solar activity and diversifying their sources of information. By gathering data from multiple channels and employing advanced prediction models, they can enhance their ability to forecast and respond to solar weather events accurately.
These prediction models can incorporate data from solar observatories, space weather monitoring satellites, and ground-based observatories, enabling a more comprehensive understanding of solar activity and its potential impact on satellite systems. In doing so, stakeholders can mitigate the risks associated with CMEs and other solar phenomena, safeguarding their satellite missions and maximising their chances of success in the challenging environment of very low Earth orbit.
India’s Entry – Aditya L1
Eight active missions are currently positioned to study the sun from different orbital regimes. Joining the journey to understand the sun is ISRO’s recent mission to study the Sun, Aditya L1. This mission entails several payloads that study different components of solar weather.
The Solar Ultraviolet Imaging Telescope (SUIT) images the Sun in Near-UV and aims at making high-cadence solar flare observations. The Visible Emission Line Coronagraph (VELC) observes the solar corona and detects the CMEs. The Aditya Solar Wind Particle Experiment (ASPEX) and Plasma Analyser Packet for Aditya (PAPA) analyse the plasma and high-energy particles associated with the solar wind.
The magnetometer performs in-situ magnetic field observations to understand the effect of the magnetic field on solar weather. The data from these payloads will provide a great understanding of solar activities and help us study and forecast solar weather.
Missions like Aditya L1 are quite important towards expanding the life span of satellites in the Earth’s orbit. The reason is that information originating from multiple sources gives multidimensional information about one subject from multiple reference points. Such information is crucial to developing and building near-to-real models to predict solar weather and its impact.
How exactly does the solar weather affect the satellites?
The satellites in orbit are subjected to specific forces, like the intensity of the Earth’s gravitational influence, third-body effects, solar radiation pressure, atmospheric drag, etc. As for the satellites closer to the Earth (Very Low Earth, Low Earth orbits), the atmospheric drag is one of the major contributing forces to the perturbations on the satellite.
The atmospheric drag is the resistance force existing due to the atmosphere above the earth extending for around 1000 km in altitude. And this drag force is not constant, as the atmosphere density that drives the drag force varies with the satellite altitude, satellite dimensions, solar weather conditions, etc. Figure 4 shows how the variations in the altitude for a combination of the ballistic coefficient of the satellite (Ratio of mass and drag area) and the solar weather conditions affect the satellite’s lifetime. A satellite’s lifetime spans from its launch into orbit until its altitude decays and falls below 200 km.
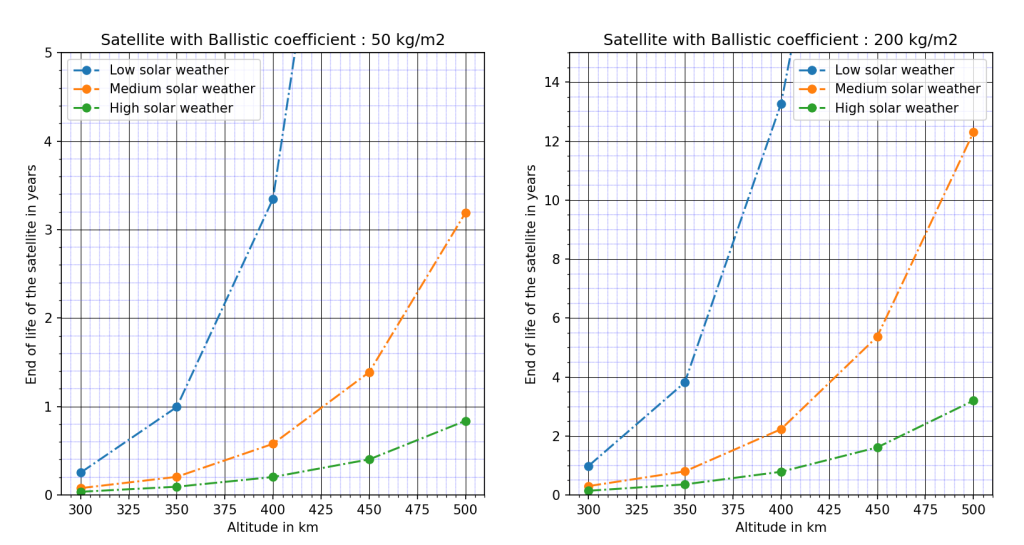
It can be observed from Figure 4 that the satellite lifetime is directly proportional to the ballistic coefficient and inversely proportional to the solar weather conditions and the altitude. The trends show that as the altitude of satellites lowers, the risk due to solar weather increases exponentially. And the low earth orbits are more prone to risk from the solar weather.
Why VLEO Despite the Risk and Threat Mitigation Strategies
A very low earth orbit is an orbit with altitude where the aerodynamic forces by the atmosphere become significant enough to affect the orbital dynamics of a satellite, typically any orbit with an altitude less than 450. VLEO orbits as we understand are greatly affected by the drag and will require regular orbit maintenance maneuvers to maintain the satellite in the operational orbit. However, despite these challenges, a VLEO EO mission has several advantages, such as,
- Increased resolution for optical payloads. For an optical EO mission, the resolution is mainly limited by the size of optics and sensor technology. Hence, reducing the distance of the target being imaged leads to an improved spatial resolution.
- Improved overall radiometric performance by suitably choosing the imaging parameters. While the ground speed increases with the reduction in orbit altitude, leading to a decreased MTF performance, the lower distance to the target being imaged increases the radiometric performance. Further, choosing the suitable parameters for the sensor, like the integration time, number of frames being added, or even freezing the ground plane for the frame time, will increase the combined MTF of the imager.
- The coverage per day is improved in the VLEO missions as the number of satellite orbits per day increases with the decreasing altitude.
- The radiation environment in a VLEO is mildly gentle compared to that in a typical LEO at 600 km. An order of magnitude reduces the peak proton flux. Hence, the part selection must be driven by the mission lifetime and need not go for radiation-hardened components.
- Space loss, also called Free Space Path Loss in the communication links, is proportional to the distance the signal should travel. The VLEO and LEO have better communication links and faster data transfer rates, facilitated by reduced space loss and minimised exposure to radiation.
- Finally, the conjunction risk is exacerbated by the increasing number of satellites in the typical LEO region of 500-800 km. A VLEO is less crowded and would require minimal fuel for a controlled deorbit at the end of the mission, leading to a sustainable space environment.
Designing an optimal VLEO EO mission involves a complex interplay between various factors, including the mission’s lifespan, satellite and sensor technology, coverage, revisit capabilities, and the cost and schedule required for satellite construction and launch. These considerations should be driven by the end-user’s requirements, which are the foundation for establishing mission objectives and identifying necessary risk mitigation strategies.
One significant challenge in VLEO EO mission design is the presence of solar weather, an inevitable peril that satellite system engineers must account for during both the mission’s design and operational phases. Despite the advantages of launching satellites into the VLEO region for EO missions, addressing solar weather’s potential risks is essential.
This includes enhancing the availability and precision of solar observation data and improving near-real-time solar weather forecasting, particularly in the lead-up to scheduled satellite launches. By proactively assessing these risks, we can mitigate the need for rapid satellite deorbiting or prevent total satellite loss. Alongside these measures, further considerations can be explored to reduce associated risks effectively.
- LEOP planning– The initial phase of the satellite mission must be meticulously planned when the satellite is not fully functional. The propulsion system will not be active during the LEOP (Launch and Early Orbit Phase). And in case of any storm, the satellite will face the brunt of the atmosphere. Hence, launching in a higher orbit than the operational orbit is recommended to complete the LEOP and calibrate the instruments. At the same time, the satellite gradually decays to the operational altitude. This will also avoid the satellite’s time being spent within the South Atlantic Anomaly, which usually results in a higher ionising dosage to the electronic components.
- Choice of propulsion system and fuel reserves – Electric propulsion systems have advantages but usually have lower “maximum thrust” ratings. This makes them less useful against any sudden increase in atmospheric drag than what the satellite would have been designed for. Hence, the propulsion system for a VLEO mission must be carefully chosen. Reserve fuel must be considered for manoeuvring during geomagnetic storms, especially for satellites in low Earth orbits, which might require propulsion to counteract increased atmospheric drag.
- Redundant or alternative system – Implement redundant critical systems so backup systems can take over if one fails due to a storm. For example, Magnetic torquers, which use Earth’s magnetic field for satellite orientation, might be less reliable during a storm, so alternatives like reaction wheels or thrusters should be available.
- Accurate Forecasting – Collaborate with agencies and institutions that monitor solar activities (like NOAA’s Space Weather Prediction Center) to receive early warnings of potential geomagnetic storms.
- Operational Protocols during Storms – It is recommended to have pre-defined operational protocols for solar storm prediction. Alter the satellite’s orientation to present a less vulnerable profile to the charged particles. Scale down or shut off non-essential systems during a storm to reduce exposure and potential damage. Move crucial data to more shielded storage areas during events. Have established protocols for potential communication disruptions, ensuring the continuity of operations even when direct communication with the satellite is intermittent or lost.
The Impact Beyond the Orbits
The impact of solar weather does not end in orbits around Earth. It extends to the surface of the Earth as well. For instance, in the fall of 1989, a geomagnetic storm caused a blackout in the province of Quebec, Canada, leaving millions without electricity. Moreover, geomagnetic storms can interfere with critical communication and navigation systems, as in the case of aviation, leading to disruption of normal operations.
This is a matter of concern, as today’s critical resources are gradually shifting to the orbits, considerably impacting the global economy regarding defence, socio-economic development and access to information.
As the world has evolved and realised the importance of the impact of space debris on satellites, it is equally important to consider the impact of solar weather. As the former is a man-made casualty, the debris movements’ impact and behaviour can be estimated. To understand and calculate the impact of solar weather, one must understand the characteristics of our star, the Sun.
It is always difficult to predict how and when the sun will attack. Because nature has never ceased to surprise humans. While we may not be able to avoid nature’s wrath entirely, we can always be prepared with diligent planning against such adverse events. Thus, as we journey further into the cosmos, understanding and preparing for both the challenges of our own making and those presented by nature becomes paramount.
For once, the famous polymath Benjamin Franklin said,
By failing to prepare, you are preparing to fail.
Authors:
- Kavya Karampuri, Mission Systems Engineer, KaleidEO
- Avinash Bagali, Product Engineer (Space Situational Awareness), KaleidEO
- SV Manoj Varma, Space Instrumentation, KaleidEO
Add comment